Computing | What Exactly Are Quantum Computers?
- Phillip Drane
- Mar 20
- 8 min read
Updated: 4 days ago
Quantum computing represents a groundbreaking frontier in computational science. It is frequently discussed yet rarely explained, partly due to its immense complexity. If you've ever wanted to understand precisely what these physicists – or that annoying co-worker – are talking about, this is the article for you.
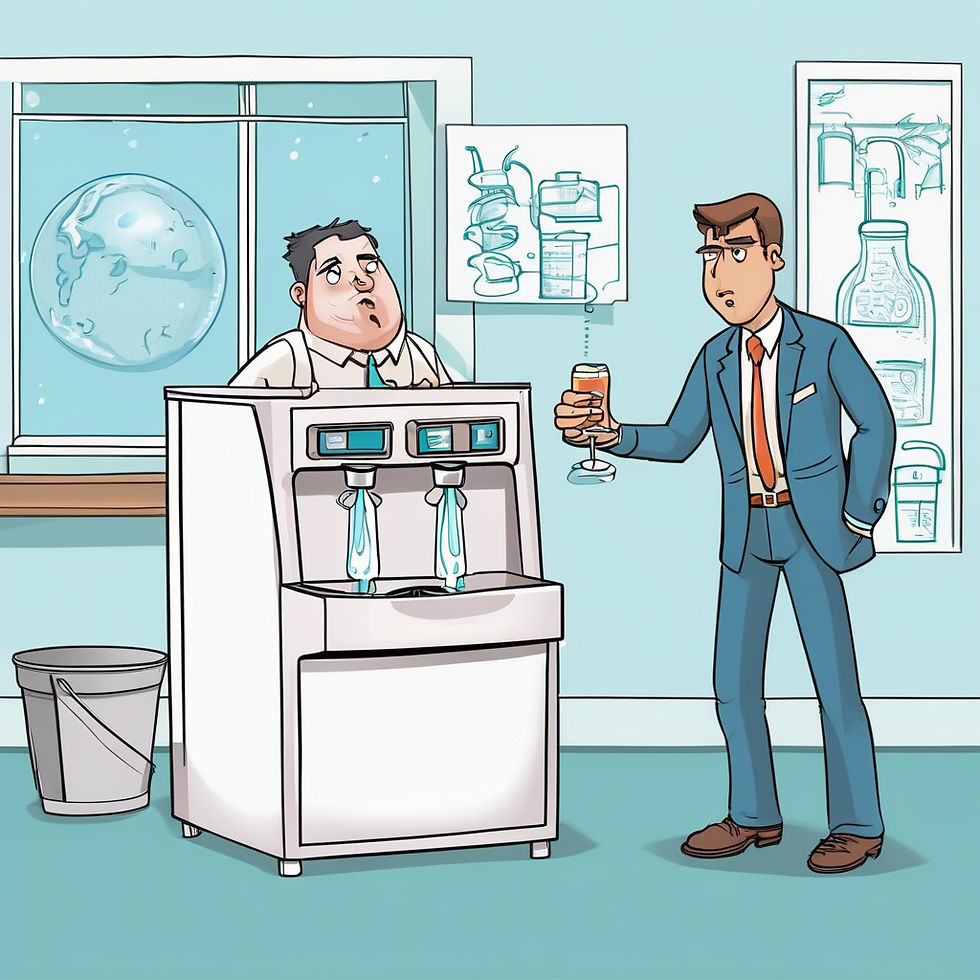
Perhaps the first thing to understand is that there isn’t just one type of quantum computer; there are many. However, these systems have several things in common: they all harness the peculiar and powerful principles of quantum mechanics to tackle problems far beyond the capabilities of their classical counterparts, and they all employ qubits (a fundamental unit of information) instead of the binary logic (0s and 1s) used in classical computing.
The unique properties of quantum computers enable exponential speed-ups for certain types of problems, making quantum computing particularly promising and a source of great excitement for scientists. But what exactly are the different types of quantum computers, and, more importantly, what advantages does each system possess for tackling specific problems?
Superconducting Quantum Computers
Are the most ubiquitous platforms to date. These systems operate at near absolute zero to achieve superconductivity in their circuits – the point at which electrical resistance completely vanishes. These circuits then form qubits, which are controlled and manipulated by microwave pulses to facilitate quantum operations.
The system is used for everything from optimisation problems in AI to quantum simulations in material science. Its almost universal adoption can be attributed to its programmability and speed, which stem from the mature and scalable nature of the platform. The platform also benefits from established semiconductor fabrication techniques and an architecture that supports a broad spectrum of quantum algorithms.
Currently, the most powerful form of this system is the Zuchongzhi 3.0. It is a 105-qubit processor that is reportedly one million times faster than Google’s Sycamore processor, which had previously claimed the title of quantum supremacy in that category. It was developed by researchers at the University of Science and Technology of China, so those stats should be taken with a grain of salt, as academia in that part of the world is known for aggrandisement.
Photonic Quantum Computers
On the other hand, these systems rely on optical components such as beam splitters, mirrors, and phase shifters to manipulate photons – the qubits in this system – and alter their quantum states. The more advanced version, which utilises quantum entanglement, requires measurement-induced nonlinearity to create the effect, as photons do not naturally interact with one another.
This system type is particularly favoured for a niche set of tasks: quantum communication, secure data transmission, and distributed quantum networks. Its suitability for these functions stems from its ability to operate at room temperature and to transmit quantum information over long distances using optical fibres. Furthermore, the system experiences reduced decoherence, as the photons travel at the speed of light.
The most powerful version of this system in Europe is the Mosaiq-12, a 12-qubit system scheduled for release in 2025. Developed by Quandela and Attocube Systems, this platform integrates a range of advanced technologies and is designed to be coupled with the Joliot-Curie supercomputer to form a hybrid HPC-Quantum platform.
Trapped Ion Quantum Computers
These systems rely on ions (charged atomic particles) that are confined and suspended in free space using electromagnetic fields within a device known as a Penning trap. Using an oscillating electrical field, these traps create a harmonic potential well, enabling the ions to form a stable linear chain. The qubits are encoded in the internal energy states of the ions – namely, their electronic or hyperfine states – which represent the quantum 0 and 1. Lasers are used to cool the ions to near absolute zero to minimise thermal motion and to manipulate their quantum states by inducing transitions between energy levels. This process enables single-qubit operations and entanglement, as the ions interact through their shared motion, also known as Coulomb interaction. The system's readout is achieved by shining a laser on the ion; its fluorescence, or lack thereof, indicates whether it is in the 0 or 1 state.
These systems are favoured for quantum error correction and simulation due to their high precision and long coherence times, which stem from the exceptional stability of the qubits and their reduced susceptibility to decoherence. Although they are not as fast as superconducting quantum computers, they are more accurate and reliable, making them the preferred choice for tasks where accuracy is prioritised over speed.
The Quantinuum H2 is currently one of the most powerful versions of this system in the world. It was developed through a collaboration between Cambridge Quantum and Honeywell Quantum Solutions. The H2 system features 32 fully connected qubits with high fidelity and long coherence times. In 2024, it achieved a 100x improvement over the existing industry benchmark.
Quantum Annealers
These specialised quantum computing systems are designed to solve optimisation problems by identifying the lowest-energy configuration of a system. They begin with qubits in a simple, low-energy state, usually a superposition of all possible states. The optimisation problem is encoded within the system's energy landscape, represented by a mathematical construct known as the Hamiltonian – a representation of the total energy of the system. The goal is to find the ground state (the lowest energy level) of this Hamiltonian, which corresponds to the optimal solution. This is achieved by gradually adjusting the strength of the quantum field, such as a transverse magnetic field. This process allows the qubits to explore the energy landscape through quantum tunnelling – a phenomenon in which energy barriers are tunnelled through rather than climbed over – thus avoiding local minima, which are states with lower energy than neighbouring states but not the lowest possible level.
This type of computational system is specifically designed for combinatorial optimisation, probabilistic sampling, and machine learning. These problems often involve large, complex search spaces with numerous local minima, which quantum tunnelling can efficiently explore to avoid becoming stuck in suboptimal solutions. Quantum annealers are, in fact, one of the few types of quantum supercomputers that are commercially available and capable of effectively handling real-world problems. The architecture used by these systems to solve problems is typically formulated as Ising models or quadratic unconstrained binary optimisation (QUBO). It is also common for these systems to be combined with classical computers to enhance problem-solving capabilities.
The most powerful quantum annealer today is the D-Wave Advantage 2. It forms part of the Advantage system, which is currently available for commercial sale and is widely utilised by industries for optimisation, machine learning, and material science applications.
Neutral Atom Quantum Computers
Neutral atoms (atoms with an equal number of protons and electrons) are utilised as qubits, leveraging the unique properties of their energy levels and interactions to enable quantum computation.
Mechanically, the process begins with atom trapping, where neutral atoms are confined using optical tweezers – tightly focused laser beams that hold the atoms in place. These tweezers can arrange the atoms into specific configurations, such as grids or arrays. The qubits are encoded in the internal energy states of the atoms, such as their hyperfine or electronic states, which represent the quantum 0 and 1. Lasers are then used to manipulate the quantum states of the atoms. Specifically, single-qubit gates are performed by applying laser pulses to induce transitions between energy levels. The computational system utilises a phenomenon called the Rydberg blockade to create entanglement between qubits. In other words, when one atom is excited to a high-energy Rydberg state, it prevents nearby atoms from being excited, enabling controlled interactions between qubits. The computation is finalised through a process known as fluorescence, where a camera captures the emitted light to determine the final states of the qubits.
The system is typically used for tasks such as quantum simulations, optimisation problems, and analogue quantum computing. Although still in relatively early stages of development, it offers significant advantages in scalability – potentially reaching thousands of qubits. Furthermore, the use of neutral atoms creates the 'ideal' qubit, as they are naturally identical and free from the risk of fabrication defects. Notably, these systems are also reconfigurable, allowing the arrangement of atoms to be dynamically adjusted.
Atom Computing’s Phoenix system is currently the most powerful of its kind. The machine utilises neutral atoms trapped in optical lattices and has achieved a record-breaking 1,180 qubits. Thanks to the inherent scalability of the system, the company plans to significantly increase the number of qubits in future iterations. The model also boasts a long coherence time, making it stable and well-suited for error correction and advanced quantum computations.
Quantum Computing Looking Ahead
Over the last few decades, progress in this field has accelerated, transforming theoretical concepts into practical implementations. Advances in manufacturing processes and increased investment have driven continual improvements in qubit coherence times, gate fidelities, and error correction techniques. These advancements have enabled the development of more reliable and scalable quantum systems, resulting in increasingly powerful processors with ever-growing qubit counts.
Perhaps the greatest indicator of how far we have progressed is the frequency with which current systems demonstrate quantum supremacy – the milestone where quantum computers surpass classical systems for specific tasks.
With AI gaining increasing importance globally and key players on the world stage striving to possess the best technology, these machines are beginning to develop the commercial market required to propel the field to the next level.
So, the question is: where do we go from here? What could the future of the field hold, and which theoretical systems are on the horizon? Could we one day see a quantum computer in every home?
Quantum Dot Computers
Nanoscale semiconductor particles, known as quantum dots, are used to confine electrons in three dimensions, creating discrete energy levels. These serve as qubits and are often referred to as artificial atoms due to their behaviour resembling that of real ones. The qubits are encoded in the spin or charge states of the electrons, with these states representing the quantum 0 and 1. The quantum states are controlled and manipulated using external electric or magnetic fields. Single-qubit operations are carried out by applying precise electromagnetic pulses to alter the spin or charge state. The quantum dots are interconnected through tunnelling and other interactions, enabling entanglement between qubits – a crucial requirement for performing multi-qubit operations and quantum computations. The system's readout is achieved by detecting changes in the electrical or optical properties of the quantum dots, with fluorescence or similar techniques used to identify the final states of the qubits.
Scientists and researchers are actively working to advance this technology due to its potential scalability and its relative ease of integration with existing semiconductor manufacturing processes. This combination makes it a more cost-effective and practical quantum computing system, with its nanoarchitecture ensuring it remains highly compact.
In response to the earlier question, if or when quantum computing enters the domestic market, this is likely the form it will take. Its compatibility with traditional circuit boards positions it as a promising candidate for hybrid quantum-classical systems. Perhaps, in the not-too-distant future, you might even be using one to read this.
Comments